![]() |
Phase-field simulations of the de-poling behavior induced by DC and AC electric fields. Credit: Nature Communications (2024). DOI: 10.1038/s41467-024-50847-3 |
Summary
1. In-device de-poling and re-poling: The researchers developed a technique to de-pole and re-pole piezoelectric materials within assembled devices using alternating current (AC) electric fields. This can be done without disassembling the device or applying heat treatment.
2. Room temperature process: Unlike conventional methods that require high temperatures (300°C or more), this new technique works at room temperature. This reduces the risk of thermal damage to other device components.
3. Reversible process: The de-poling and re-poling process is reversible and can be repeated multiple times without degradation in properties. This allows for multiple "revivals" of a device's performance over its lifetime.
4. Restoration of device functionality: For ultrasound transducers used as a test case, the electrical impedance/phase spectra and pulse/echo response could be revived after each re-poling cycle. This suggests that devices can be restored to full functionality even after partial de-poling due to use or environmental factors.
5. Controlled de-poling: The AC electric field method allows for more controlled de-poling compared to DC fields, which only induce a transient de-poled state. This gives engineers more precise control over the piezoelectric material's state.
6. Potential for in-situ maintenance: While not explicitly stated, the room-temperature, in-device nature of this technique suggests potential for developing in-situ maintenance procedures for piezoelectric devices in the field.
7. Extended device lifespan: By providing a way to repeatedly restore the piezoelectric properties of materials within devices, this technique could significantly extend the operational lifespan of piezoelectric devices.
8. Reduced waste: The ability to restore device functionality without replacement of the piezoelectric component could reduce electronic waste associated with these devices.
9. New design possibilities: This technique may allow engineers to design piezoelectric devices with easier access for maintenance, knowing that the critical poling step can be performed in-situ.
Overall, this research provides a powerful new tool for maintaining and restoring piezoelectric devices, potentially leading to more durable, longer-lasting, and easier-to-maintain piezoelectric technologies in fields like medical imaging, sonar, and various sensor applications.
Authors
1. Hwang-Pill Kim, Huaiyu Wu, Zhengze Xu, Sipan Liu, Sunho Moon, Jong Eun Ryu, Jun Liu, and Xiaoning Jiang are from the Department of Mechanical and Aerospace Engineering at North Carolina State University.
2. Mao-Hua Zhang and Long-Qing Chen are from the Department of Materials Science and Engineering at Pennsylvania State University.
3. Bo Wang is from the Materials Science Division at Lawrence Livermore National Laboratory.
4. Yohachi Yamashita has dual affiliations:
- Department of Mechanical and Aerospace Engineering, North Carolina State University
- Department of Human and Environmental Science, Shonan Institute of Technology, Japan
5. Shujun Zhang is from the Institute for Superconducting and Electronic Materials, Faculty of Engineering and Information Sciences, University of Wollongong, Australia.
Contributions:
- H.P. Kim, L.Q. Chen, Y. Yamashita, and X. Jiang conceived and planned the study.
- H.P. Kim, H. Wu, S. Moon, Z. Xu, S. Liu, and Y. Yamashita performed the experiments.
- M.H. Zhang, B. Wang, and L.Q. Chen performed the simulations.
- H.P. Kim, Z. Xu, S. Liu, Y. Yamashita, J.E. Ryu, J. Liu, and S. Zhang performed data analysis.
- H.P. Kim, H. Wu, M.H. Zhang, L.Q. Chen, J.E. Ryu, J. Liu, and X. Jiang wrote the paper.
Researchers solve long-standing challenge for piezoelectric materials
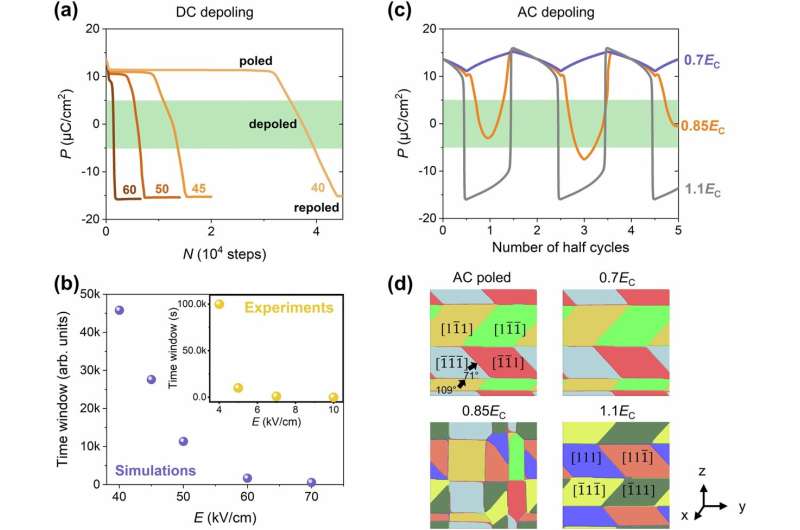
Heat and pressure can deteriorate the properties of piezoelectric materials that make state-of-the-art ultrasound and sonar technologies possible—and fixing that damage has historically required disassembling devices and exposing the materials to even higher temperatures.
Now researchers have developed a technique to restore those properties at room temperature, making it easier to repair these devices—and paving the way for new ultrasound technologies.
The paper, "Electrical De-poling and Re-poling of Relaxor-PbTiO3 Piezoelectric Single Crystals without Heat Treatment," is published in Nature Communications.
Piezoelectric materials have many applications, including sonar technologies and devices that generate and sense ultrasound waves. But for these devices to efficiently generate sonar or ultrasound waves, the material needs to be "poled."
That's because the piezoelectric materials used for sonar and ultrasound applications are mostly ferroelectric. And like all ferroelectric materials, they exhibit a phenomenon called spontaneous polarization. That means they contain pairs of positively and negatively charged ions called dipoles.
When a ferroelectric material is poled, that means all of its dipoles have been pulled into alignment with an external electric field. In other words, the dipoles are all oriented in the same direction, which makes their piezoelectric properties more pronounced.
"If those dipoles aren't in alignment it's difficult to generate targeted ultrasound waves with the amplitude needed for them to be practical," says Xiaoning Jiang, corresponding author of a paper on the work and Dean F. Duncan Distinguished Professor of Mechanical and Aerospace Engineering at North Carolina State University.
"Preserving the poling of piezoelectric-ferroelectric materials poses some significant challenges, because the dipoles can begin losing their alignment when exposed to elevated temperatures or high pressures," Jiang says.
"This is also a manufacturing problem, because it limits which other materials and processes you can use when making ultrasound devices," Jiang says. "And because the elevated temperatures aren't even really that high—you can see alignment problems as low as 70°C—even shipping or storing these technologies can sometimes adversely affect the poling and the efficiency of the devices.
"What's more, extended use of some technologies can result in the device itself generating heat that risks depoling the piezoelectric-ferroelectric material."
And once the dipoles in the material have come out of alignment, getting them back into alignment isn't easy. The piezoelectric-ferroelectric material needs to be removed from the device and exposed to high heat—300°C or more—in order to completely depole the material before "repoling" it and pulling the dipoles back into alignment.
"It's important to re-use these piezoelectric-ferroelectric materials because they are usually expensive—you don't want to just throw them away," Jiang says. "But often the material is retrieved and the rest of the ultrasound device is discarded.
"We have developed a technique that allows us to depole and repole piezoelectric-ferroelectric materials at room temperature. That means we can pull the dipoles back into alignment without removing the material from the device—and this can be done repeatedly, as needed."
To understand the new technique, you need to understand that there are two ways to pull the dipoles in a piezoelectric-ferroelectric material into alignment. The most widely used technique involves applying a direct current (DC) electric field to the material, which pulls all of the dipoles in the same direction.
"This way works well for creating alignment, but it is virtually impossible to depole the material using only a DC field," Jiang said.
The other technique involves applying an alternating current (AC) electric field to the material, which causes the dipoles to oscillate in response to the waves in the field, until the field is removed, at which point the dipoles lock into place in alignment.
"We found that we can also depole the material using an AC field, even at room temperature. If the material was originally poled using a DC field, we could remove much of the poling with an AC field—but not all of it," Jiang said. "However, if the material was originally poled with an AC field, we found that could also completely depole the material using an AC field."
The finding has at least two significant ramifications for ultrasound technologies.
"If we can pole piezoelectric-ferroelectric materials at room temperature, it means we can alter the other materials and manufacturing processes we use when creating ultrasound devices to optimize their performance," Jiang says. "We are no longer limited to materials and processes that won't affect the polarization in the piezoelectric-ferroelectric components, because we can pole the material using an AC field after the device has been assembled.
"What's more, it means that we can easily repole the materials in existing devices, hopefully giving us a long lifetime of peak performance for these technologies."
More information: Hwang-Pill Kim et al, Electrical de-poling and re-poling of relaxor-PbTiO3 piezoelectric single crystals without heat treatment, Nature Communications (2024). DOI: 10.1038/s41467-024-50847-3
Citation: Researchers solve long-standing challenge for piezoelectric materials (2024, August 6) retrieved 10 August 2024 from https://phys.org/news/2024-08-piezoelectric-materials.html
This document is subject to copyright. Apart from any fair dealing for the purpose of private study or research, no part may be reproduced without the written permission. The content is provided for information purposes only.
Electrical de-poling and re-poling of relaxor-PbTiO3 piezoelectric single crystals without heat treatment
Introduction
Relaxor-PbTiO3 (PT) ferroelectric single crystals such as Pb(Mg1/3Nb2/3)O3-PbTiO3 (PMN-PT) and Pb(In1/2Nb1/2)O3-Pb(Mg1/3Nb2/3)O3-PbTiO3 (PIN-PMN-PT) have been on the forefront in electromechanical applications including actuators and transducers due to their outstanding piezoelectric and dielectric properties, compared to the current existing ceramic counterparts1,2,3. To meet the increasing demands on high-performance devices, much effort has been devoted to further improve their properties via compositional tuning4. Nevertheless, the relatively low coercive fields (e.g., <7 kV/cm) and phase transformation temperatures (e.g., <125 °C) of relaxor-PT single crystals deteriorate the polarization of crystals exposed to certain electrical, thermal, and stress fields due to the partial or full de-poling during the device applications or manufacturing5,6,7,8,9. Given that high piezoelectricity in devices is only available when ferroelectric domains are properly aligned into a preferred direction, poling is indispensable for ferroelectrics-based piezoelectric materials for device fabrications10. However, undesired de-poling is encountered when a phase transformation or domain switching occurs during operations, which cancels out net macroscopic piezoelectricity and ferroelectricity11,12. In specific, heat generation during device operations is definitely unfavorable because phase transformations can potentially occur at elevated temperatures13. It is well accepted that de-poling of relaxor-PT single crystals is initiated at their first phase transformation temperature during heating, usually rhombohedral-to-tetragonal phase transformation temperature (TR-T)14,15. If any retaining force is absent, at least a partially-depoled state will be formed when a temperature passes through phase transformation temperatures. To solve the aforementioned issue, TR-T has been continuously improved by designing ternary single crystals or incorporating dopant elements into relaxor-PT single crystals at the expense of electromechanical responses16,17. Poled states are also vulnerable to external electric fields18,19. Once the applied field deviating from the initial poling direction approaches the coercive field (Ec), not only electromechanical responses but also impedance spectra are likely deteriorated due to de-poling20,21, indicating that ferroelectric materials should be repoled for normal device operations. Conventionally, a thermal treatment is necessary to fully depole the partially depoled piezoelectric materials, followed by a re-poling process. However, the thermal treatment process usually involves a temperature above the Curie temperature (Tc), which is not tolerable for most assembled piezoelectric devices. Therefore, a study on the revival of partially depoled in-device ferroelectrics via a fully de-poling and re-poling process without thermal annealing is significant since degraded polarizations directly lead to the malfunction of devices. The findings from this work pave a way toward tailoring a poled state of active piezoelectric materials in electronic devices without thermal treatments.
Results
De-poling by external electric fields
It is well known that electric fields opposite to a poling direction likely result in domain switching or induce domain nucleation and growth22. Hence, the switching of aligned (poled) domains and domain realignment are usually expected in the case that a direct current (DC) electric field is applied against an initial polarity of poled states, and no polarity switching is expected if the DC electric field is applied along the initial polarity (Fig. S1). However, how electric fields with different waveforms impact poled states has not been systematically studied. In spite of the fact that alternating current (AC) poling can generate different domain configurations with enhanced piezoelectric and dielectric properties compared to direct current (DC) poling23,24, the way AC electric fields impact aligned domains has not been well investigated. We hypothesize that AC electric fields may induce different de-poling kinetics due to their bidirectional switched polarity compared to DC electric fields, whose polarity is unidirectional. Here, we studied the de-poling of initially AC-poled (ACP) PIN-PMN-PT single crystals and DC-poled (DCP) single crystals by applying external AC electric fields and DC electric fields, respectively.
ACP and DCP crystals were investigated to see how poled states deteriorate in response to applied external AC electric fields (Figs. 1 and 2). We first obtained the properties of ACP and DCP crystals. Their optimum properties (ACP: dielectric constant = 6170 ± 220 and piezoelectric coefficient = 1820 ± 40 pC/N; DCP: dielectric constant = 4630 ± 100 and piezoelectric coefficient = 1400 ± 50 pC/N) are denoted in the figures for better comparisons. The peak amplitudes, cycles, and frequencies of the applied external AC electric fields were varied, and the de-poling of the poled crystals was assessed by measuring the dielectric property, piezoelectric property, and the impedance/phase spectrum. Figure 1b, c present variations in dielectric constants and piezoelectric coefficients of the ACP crystals after the poled crystals were exposed to external AC electric fields. When the peak amplitude (2 kV/cm) was less than the crystals’ Ec (4.8 kV/cm), the polarization direction did not change, and hence, the initial ACP crystal properties were maintained regardless of cycles and frequencies of the external AC electric fields. As supported by the impedance spectrum (Fig. 1d), de-poling does not occur at subthreshold driving electric fields because of the poor polarization polarity switching below Ec.
a Schematic illustrations of experimental procedures for PIN-PMN-PT single crystals that were first poled by AC electric fields and then depoled by AC electric fields. b Dielectric constants, (c) piezoelectric coefficients, and (d) impedance spectrum of the PIN-PMN-PT single crystals after applying AC electric fields for de-poling. Error bars indicate the sample standard deviation of n = 3 measurements carried out on independent samples. The peak amplitudes, cycles, and frequencies of the AC electric fields were varied to investigate how poled states change in response to external electric fields. Compared to the de-poling by DC electric fields (Fig. S2), AC electric fields can readily induce an almost completely depoled state by simply adjusting the amplitude and frequency of AC electric fields.
a Schematic illustrations of experimental procedures for PIN-PMN-PT single crystals that were first poled by DC electric fields and then depoled by AC electric fields. b Dielectric constants, (c) piezoelectric coefficients, and (d) impedance spectrum of the PIN-PMN-PT single crystals after applying AC electric fields for de-poling. Error bars indicate the sample standard deviation of n = 3 measurements carried out on independent samples. The peak amplitudes, cycles, and frequencies of the AC electric fields were controlled to investigate how poled states are destabilized in response to external electric fields. Similar to the DC electric field for de-poling (Fig. S3), initial poled states are influential in conditions for de-poling.
On the other hand, in case the peak amplitude (4 kV/cm) of the AC electric fields for de-poling was comparable to their Ec (4.8 kV/cm), the dielectric and piezoelectric properties of the crystals were unchanged at higher frequencies (≥103 Hz), but significant decreases in both properties were detected at lower frequencies (≤10 Hz). This can be explained in such a way that domain switching can hardly be completed at high frequencies, but domains can be switched back and forth at low frequencies, resulting in de-poling. With much lower dielectric constants than unpoled crystals, the impedance spectrum of the depoled crystals also turns flat as unpoled crystals. In case the peak amplitude (8 kV/cm) was higher than Ec, the crystals tended to be repoled during the domain switching process. As a result, the degrees of de-poling were overall smaller than those of de-poling induced by the external AC electric field with a lower peak amplitude of 4 kV/cm since re-poling cannot be completed at electric fields close to Ec. Therefore, an AC electric field with an amplitude comparable to Ec is the key to obtaining a fully depoled state of initially ACP crystals. For initially DCP crystals (Fig. 2), de-poling was initiated at higher peak amplitudes as their Ec is higher than that of ACP crystals (Figs. S4a, S4b). Decreased dielectric and piezoelectric properties were observed at low frequency AC electric fields with amplitudes of 5–8 kV/cm (Fig. 2b, c). However, the electrical impedance spectrum (Fig. 2d) still showed multiple resonance modes, suggesting a partial de-poling process. Furthermore, the re-poling was more obvious at peak amplitudes surpassing Ec because initially DCP crystals were repoled with enhancements by AC electric fields. Consequently, initially DCP crystals were less depoled than initially ACP crystals. In short, the degrees of de-poling by AC electric fields depend on initial polarization conditions. The results indicate that a poled state can be switched into a fully depoled state without thermal annealing above their Tc, which suggests a significant advantage in device applications. Given that heat treatments are certainly unfavorable once piezoelectric materials are fabricated into devices, this finding is significant in controlling a poled state of active piezoelectric materials in devices when they become inactive by external stimuli such as mechanical stress or heat generations during operations or manufacturing processes.
To track changes in piezoelectric and dielectric properties of single crystals during de-poling triggered by AC electric fields, we measured in-situ electric field induced dielectric constants and piezoelectric strain coefficients at each electric field cycle (Fig. 3). Here, the amplitude and frequency of the AC electric field for de-poling was 4 kV/cm and 1 Hz, respectively. At the 1st AC electric field cycle, the properties of the initially ACP crystals were significantly degraded. Even after the 5th and 10th cycles, the depoled properties were maintained. During the de-poling process, the polarization and electric field-induced currents were also varied (Figs. S4c, S4d, S5), implying that ferroelectric states were changed. These results indicate not only poling but also de-poling can be controlled by external AC electric fields. Therefore, we expect that the proposed method using AC electric fields can also be applied to ferroelectrics-based devices to manipulate ferroelectric states.
a dielectric constants response and (b) piezoelectric coefficients response. The peak amplitude and frequency of the AC electric fields for de-poling were 4 kV/cm and 1 Hz, respectively. Variations in the properties were measured at each cycle. The results show that the poled crystals were clearly depoled after AC electric fields were applied.
Reversible de-poling and re-poling process
To confirm its reproducibility, poling and de-poling induced by electric fields were sequentially conducted (Fig. 4a). Figure 4b, c provide dielectric constants and piezoelectric coefficients after multiple poling and de-poling processes, respectively. Here, the optimized ACP condition was used for the poling, while a peak amplitude of 4 kV/cm, 10 Hz, and 10 cycles were adopted for the de-poling. It can be observed that the depoled state triggered by AC electric fields can repeatedly return to the poled state without discrepancies in properties, and vice versa, implying that these polarization switching processes are reversible without the conventional annealing process. Moreover, the impedance spectrum of a crystal after the 5th de-poling process was still similar to that of an unpoled crystal with significantly suppressed resonance modes (Fig. 4d). These results demonstrate the feasibility of relaxor-PT single crystals to be repeatedly depoled and repoled by applying external AC electric fields without conventional thermal annealing and device disassembly. Similar experiments were conducted by applying external DC electric fields to depolarize relaxor-PT single crystals, and only a transient depoled state was observed within a fraction of 1 s time (Fig. S2), suggesting non-practical controls in DC electric fields induced de-poling processes.
a Schematic illustrations of repetition tests with multiple poling and de-poling processes that were conducted on the PIN-PMN-PT single crystals. Here, the optimized ACP condition (a peak amplitude of 10 kV/cm, 20 cycles and 30 Hz) was used for the poling while a peak amplitude of 4 kV/cm, 10 cycles and 1 Hz were adopted for the de-poling. b Dielectric constants and (c) piezoelectric coefficients of the PIN-PMN-PT single crystals during multiple poling and de-poling processes to confirm the reproducibility of the de-poling induced by AC electric fields. d Impedance spectrum of crystals after multiple poling and de-poling processes to ensure its effectiveness.
Phase-field simulations on electric fields induced de-poling
Phase-field simulations were performed to understand the de-poling behavior under AC and DC electric fields. The unpoled piezoelectric sample with random polarization directions was first poled using AC electric fields to ensure a poled state (Fig. S6). For the DC electric field induced de-poling, the sample was subsequently exposed to DC electric fields in the opposite direction to the original poling direction. The time dependence (represented by the number of simulation steps) of poled states of the investigated sample is shown in Fig. 5a, where the sample first undergoes de-poling and then becomes repoled in the opposite direction. Here, de-poling is defined as when the macroscopic polarization falls within ±5 μC/cm2, highlighted by the green area. The time difference between the two intersections of the polarization curve with the green range is considered as the time window for ideal de-poling of the sample. As illustrated in Fig. 5b, the higher the amplitude of the applied electric fields, the narrower the time window to ensure ideal de-poling. On the one hand, the use of high DC electric fields easily leads to re-poling of the sample and is thus uncontrollable. On the other hand, despite the wider time window at low DC electric fields, this is kinetically and experimentally impractical because it requires significantly more time. For example, the time required to depolarize a sample is ~1000 times longer when the applied field slightly decreases from 5 kV/cm to 4 kV/cm (Fig. 2c).
a Time dependence, represented by the number of simulation steps, of the polarization of the investigated piezoelectric sample exposed to DC electric fields of 40, 45, 50, and 60 kV/cm. Note that the applied fields in the phase-field simulations are significantly larger than experimental values since the nucleation of domains at crystal defects in real ferroelectric materials lowers the actual coercive field. b Simulated and experimentally determined time window to ensure de-poling using DC electric fields, defined when the polarization magnitude is <5 μC/cm2. The time difference between the two intersections of the polarization curve with the green “de-poling region” is defined as the time window. c Polarization as a function of the number of cycles of the investigated sample exposed to AC electric fields of 0.7Ec, 0.85Ec, and 1.1Ec. d The domain structures of the ACP sample and the final states of the sample subjected to different AC electric fields. The domains with identical polarization vectors have the same colors, and their polarization vectors are labeled.
For the AC electric field induced de-poling, the sample in the poled state was exposed to AC electric fields and the poled states changed as a function of the number of cycles as shown in Fig. 5c. At 0.7Ec, de-poling is insignificant and can be neglected. At 1.1Ec, the sample easily becomes repoled, and it is difficult to obtain the depoled state. At 0.85Ec, an electric field slightly lower than the coercive field, de-poling of the sample is readily realized in a controlled manner, which agrees well with the experimental results (Fig. 1). The simulated domain structures of unpoled (Fig. S6), AC poled and AC depoled states under different electric fields (0.7 Ec, 0.85 Ec, and 1.1 Ec) are shown in Fig. 5d. The process of the AC de-poling at 0.85Ec is illustrated in Supplementary Movie 1. The influence of frequencies on the de-poling behavior is shown in Fig. S7, where either a high or a low frequency results in an unstable depoled state, while a medium frequency can realize a completely depoled state.
To confirm our phase-field simulation results, we visualized the ferroelectric domains of the single crystals using piezoresponse force microscopy (PFM) (Fig. S8). The domain configurations were captured at different poling states for better comparisons. In contrast with ACP which generates periodic domain structures, de-poling by AC electric fields induces complex structures which are similar to DCP. Further, the simulation shows that de-poling by AC electric fields also leads to DCP-like irregular patterns. In this regard, our simulation results are consistent with the experimental results. In addition, we also measured the X-ray diffraction patterns of the crystals to track crystallographic changes during de-poling by AC electric fields (Fig. S9). When the initial poling state is ACP (ACP-EDP), the diffraction pattern after de-poling is shifted to a lower angle and consequently similar to DCP. This supports our argument from the simulation that de-poling by AC electric fields results in DCP-like domain configurations.
In-device de-poling and re-poling without heat treatment
We then investigated AC electric field induced de-poling and re-poling feasibility for PIN-PMN-PT single crystals in an assembled ultrasound transducer device (Figs. S10, S11, S12 and S13). The results show that active piezoelectric materials in devices can be truly depoled and repoled using AC electric fields without heat treatments or device disassembly, and the electrical impedance/phase spectra (Fig. S10) and pulse/echo response (Fig. S11) of the device can be revived after each re-poling. In contrast, the ultrasound transducer cannot function properly after de-poling and re-poling induced by DC electric fields (Figs. S12, S13).
Up to this point, we demonstrate that ACP PIN-PMN-PT single crystals can be depoled and repoled without thermal treatment, which is significantly beneficial for active piezoelectric materials in devices with the adjustable ferroelectric state when piezoelectric materials are partially depoled. To obtain a fully depoled state, conditions for AC electric fields should meet some requirements. (1) For the amplitude, it should be enough to initiate domain nucleation and growth but less than substantial amplitudes (~1.5 times Ec) to avoid electric re-poling. (2) In the case of (001)- oriented PIN-PMN-PT single crystals with the dimension of 3 × 3 × 1.0 mm3, the frequency of electric fields should be <10 Hz to achieve a completely depoled state. Although the reason why low frequencies are required is still under discussion, it can be speculated that polarization relaxation originated by depolarization fields may determine optimized frequencies for a fully depoled state. This is why the same frequency ranges for de-poling may not be applied to other types of relaxor-PT single crystals and polycrystalline ceramics, implying that optimum frequency ranges are dependent on materials and dimensions. To confirm its feasibility for other piezoelectric ceramics, we conducted the proposed de-poling experiments on Pb(Zr,Ti)O3 (PZT) polycrystalline ceramics (Fig. S14). The dimension of the ceramics is similar to that of the crystal in this work, and the amplitude of AC electric fields for de-poling is determined based on their Ec. Compared to PIN-PMN-PT single crystals, prominent de-poling behaviors were not observed in the PZT samples under the selected experimental conditions. Therefore, we can deduce that the optimized conditions for de-poling may be highly dependent on the types of materials. Further investigations are needed to study strategies to find optimum de-poling conditions for other types of piezoelectric ceramics.
We expect the suggested idea will be useful thanks to its capabilities in achieving desired ferroelectric states; the proposed method can depolarize ferroelectric materials and then achieve a desired polarization state without heat treatment and device disassembly. In this regard, the advantage of our idea is the tunability of ferroelectric materials inside piezoelectric devices, which is usually impossible so far. The idea can be also extended to ferroelectrics-based devices. More ferroelectric variants in ferroelectrics-based memory devices are definitely beneficial for next-generation applications to achieve new functionality. Therefore, investigating how to precisely control ferroelectric states is our principal goal for future works.
Discussion
We investigated the de-poling and re-poling of poled PIN-PMN-PT single crystals under DC and AC electric fields. We found that low-frequency AC electric fields with peak amplitudes comparable to Ec can almost completely depolarize the crystals, and the resultant piezoelectric properties are comparable to those of unpoled crystals. With all vibration modes suppressed, the proposed method can be used as an alternative to the conventional thermal de-poling approach for piezoelectric materials. Furthermore, the crystal depoled by AC electric fields in actual devices can be poled again without degradation in properties. The phase-field simulations confirmed our experimental results that AC electric fields can depolarize the crystals in a controllable manner, while DC electric fields only induce a transient depoled state over a short period of time (a fraction of 1 s). The current findings provide not only an insight toward the stabilities of poled states of relaxor-PT single crystals under external electric fields but also a potential method to control a poled state of ferroelectric materials in devices.
Methods
Sample preparation
(001)- oriented PIN-PMN-PT single crystals (CTS Inc., IL, USA) were prepared and diced into kt mode (3 × 3 × 1.0 mm3) with Ti/Au electrodes (10/200 nm) on the principal surfaces. Electric poling, including DCP and ACP, was performed at room temperature (25 °C) using a function generator (Agilent Technologies, 33250 A, Santa Clara, CA, USA) and a high voltage amplifier (Trek, 609B, Sausalito, CA, USA) in a customized fixture filled with silicone oil. Before poling, all crystals were annealed at 250 °C for 15 min to ensure a completely depoled state. For DCP, the optimized electric field with an amplitude of 10 kV/cm was applied for 300 s. For ACP, bi-polar triangular electric field (peak amplitude of 10 kVpp/cm, energetically equivalent to 5.8 kVrms/cm) was applied with 20 cycles at 30 Hz25,26. To confirm the feasibility of the proposed idea for polycrystalline ceramics, Pb(Zr,Ti)O3 ceramics (CTS Inc., IL, USA) were prepared. The dimension of the ceramic samples is 3 × 3 x 0.9t mm3, which is similar to that of the crystals in this work. The schematic illustrations depicting the experimental setup and characterizations are provided in Fig. S15.
Characterizations
The piezoelectric coefficients (d33) and dielectric constants (εT33/ε0) of poled crystals and ceramics were measured using a quasi-static piezo d33 meter (Chinese Academy of Science, Model ZJ-4B, China) and a multi-frequency LCR meter (Agilent Technologies, 4294 A, Santa Clara, CA, USA) at 1 kHz, respectively. To depolarize poled crystals and ceramics using external electric fields, both DC and AC electric fields were applied using the same equipment. The DC electric fields for the de-poling were applied along and opposite to the polarity determined by the original polarization, and their amplitude and duration time were controlled. Likewise, the de-poling triggered by AC electric fields was conducted with different amplitudes, cycles, and frequencies. To assess how poled crystals are indeed depoled by the external electric fields, their piezoelectric coefficients and dielectric constants were measured together with impedance spectra. Polarization hysteresis loops, current curves, and in-situ electric field induced piezoelectric strain coefficients and dielectric constants were measured by a ferroelectric measurement system (aixPES, aixACCT, Aachen, Germany). X-ray diffraction patterns of the crystals at different poling states were obtained using a PANalytical EMPYREAN diffraction instrument (Malvern Panalytical Inc., Westborough, MA, USA) with Cu-Kα radiation. To observe domain structures of the crystals after poling and de-poling, their fractured surfaces parallel to the electrode were scanned using piezoresponse force microscopy (PFM) (Bruker, Dimension Icon, Santa Barbara, CA, USA).
Phase-field simulations
The formation and evolution of domain structures under the DC and AC electric fields were simulated with the phase-field method. In the phase-field model, the total polarization, P, is used as the order parameter to describe the temporal evolution of the system based on the time-dependent Ginzburg-Landau (TDGL) equation27:
$$\frac{\partial {{{\bf{P}}}}{{{\boldsymbol{(}}}}{{{\bf{r}}}}{{{\boldsymbol{)}}}}}{\partial t}=-L\frac{\delta F}{\delta {{{\bf{P}}}}{{{\boldsymbol{(}}}}{{{\bf{r}}}}{{{\boldsymbol{)}}}}}$$
(1)
where L is the kinetic coefficient, r is the space position, t is the time, and F is the total free energy of the system, including the contributions of the bulk, electrostatic, elastic, and gradient energies (F = Fbulk + Felectrostatic + Felastic + Fgradient). The detailed expressions of the above free energies can be found elsewhere27,28. The Landau coefficients were adapted from Ref. 29 and are based on the characterization of the electrical properties of PMN-PT single crystals at room temperature. A complete set of the material properties used in this work was employed elsewhere30 and is given in Supplementary Table 1. Phase-field simulations were performed in a quasi-two-dimensional (2D) system discretized into 512Δx × 512Δx × 2Δx grid points with a grid size Δx = 0.5 nm. The electrostatic and elastostatic equilibrium equations were solved using the Fourier spectral method31. When solving the equations, the three-dimensional periodic boundary condition is imposed. For the elastostatic equation, the average strain of the simulated system is fixed to be zero at all time steps, corresponding to a constrained mechanical boundary condition. For the electrostatic equation, the average electric field of the system is maintained to be equal to the applied electric fields for ACP, DCP and de-poling processes which vary as a function of time. The contribution from the external electric field is included in the electrostatic energy,
$${F}_{{\mbox{electric}}}=\int (-\frac{1}{2}{E}_{i}^{{{\mathrm{int}}}}{P}_{i}-{E}_{i}^{{ext}}{P}_{i}){dV}$$
(2)
where Eint and Eext denote the internal field and the external electric field, respectively. The external field Eext is equal to the applied time-dependent AC or DC electric fields. The internal field Eint is then solved through the following electrostatic equation:
$${\kappa }_{0}{\kappa }^{b}{{{\boldsymbol{\nabla }}}}{{{{\bf{E}}}}}^{{{{\bf{int}}}}}+{{{\boldsymbol{\nabla }}}}{{{\bf{P}}}}=0$$
(3)
where κ0 and κb are the vacuum permittivity and background dielectric constants, respectively. The unpoled domain structure was obtained by relaxing the system from a random noise distribution of polarization. Subsequently, a low-frequency triangular wave electric field with an amplitude (93 kV/cm) larger than the coercive field was applied along the y direction of the system to obtain the poled state. Afterwards, the AC-poled state was subjected to AC and DC electric fields to study the de-poling behavior. For the DC de-poling, samples were exposed to DC electric fields of varying strength opposite the polarity direction. For the AC de-poling, samples were exposed to AC electric fields of varying amplitudes.
Feasibility test of de-poling and re-poling for piezoelectric materials in devices
For in-device de-poling, where the piezoelectric components were partially depoled because of the exposure to the thermal, stress, or electric fields during the device manufacturing and applications, both ACP/DCP samples were prepared as pre-mentioned. First, two PIN-PMN-PT samples with a thickness of 1 mm and an aperture of 3 × 3 mm2 were prepared with the AC/DC polarization method, respectively. Acoustic impedance Z33 = 4 MRayls of Al2O3/epoxy was chosen as the matching layer and was attached on the front of the transducer with a thickness control of 350 μm. Then, the conductive backing (Roll Inc., Cleveland, OH, USA) with Z33 = 6 MRayls was bonded to the transducer with a thickness of 500 μm. After that, a 20 cm-long coaxial cable was connected to the transducer with silver epoxy (E-solder 3022, Von-Roll Inc., Cleveland, OH, USA) with low resistance (<1 Ω). Then, the whole structure was then covered with thin UV curing epoxy (20 μm) as the passivation layer to prevent corrosion or chemical reactions with the surrounding environment. Then, the device was first characterized with an impedance analyzer (4294 A, Agilent Tech. Inc., Santa Clara, CA, US) for the electrical impedance and dielectric loss. Afterward, a pulse/echo test was conducted in a deionized degassed water tank at room temperature. The transducer was excited by a pulser/receiver (5900 PR, Olympus, WA, USA) with a PRF of 200 Hz and pulse voltage of 200 V without gain. A steel bar was used as the reflector at a distance of 18 mm. The RF signal was collected with the oscilloscope (DSO7104B, Agilent Technologies, Santa Clara, CA, USA). The measured pulse-echo signal was used to obtain the bandwidth of the prototyped transducers. Performance after the first AC/DC poling was used as the reference for transducers. Then, for each transducer, two repetitions were conducted with the de-poling and re-poling process. The electrical impedance and pulse/echo performance tests were conducted for comparison at each step.
Data availability
The authors confirm that all data generated or analyzed during this study are included in this article and supplementary information files and are available from the corresponding author (X.J.) on request.
References
Park, S. & Shrout, T. R. Ultrahigh strain and piezoelectric behavior in relaxor based ferroelectric single crystals. J. Appl. Phys. 82, 1804–1811 (1997).
Zhang, S. & Li, F. High performance ferroelectric relaxor-PbTiO3 single crystals: Status and perspective. J. Appl. Phys. 111, 031301 (2012).
Zhang, S. et al. Advantages and challenges of relaxor-PbTiO3 ferroelectric crystals for electroacoustic transducers–a review. Prog. Mater. Sci. 68, 1–66 (2015).
Li, F. et al. Giant piezoelectricity of Sm-doped Pb (Mg1/3Nb2/3) O3-PbTiO3 single crystals. Science 364, 264–268 (2019).
Yamashita, Y. et al. A review of lead perovskite piezoelectric single crystals and their medical transducers application. IEEE Trans. Ultrason. Ferroelectr. Freq. Control 69, 3048–3056 (2022).
Kim, H. et al. Strategies of a potential importance, making lead-free piezoceramics truly alternative to PZTs. J. Korean Ceram. Soc. 54, 86–95 (2017).
Zhang, S. et al. Field stability of piezoelectric shear properties in PIN-PMN-PT crystals under large drive field. IEEE Trans. Ultrason. Ferroelectr. Freq. Control 58, 274–280 (2011).
Sapper, E., Schaab, S., Jo, W., Granzow, T. & Rödel, J. Influence of electric fields on the depolarization temperature of Mn-doped (1-x) Bi1/2Na1/2TiO3-xBaTiO3. J. Appl. Phys. 111, 014105 (2012).
Su, S. et al. Poling dependence and stability of piezoelectric properties of Ba (Zr0.2Ti0.8) O3-(Ba0.7Ca0.3) TiO3 ceramics with huge piezoelectric coefficients. Curr. Appl. Phys. 11, S120–S123 (2011).
Damjanovic, D. Ferroelectric, dielectric and piezoelectric properties of ferroelectric thin films and ceramics. Rep. Prog. Phys. 61, 1267 (1998).
Gehring, P. M., Ohwada, K. & Shirane, G. Electric-field effects on the diffuse scattering in PbZn1∕ 3Nb2∕ 3O3 doped with 8% PbTiO3. Phys. Rev. B 70, 014110 (2004).
Hou, D. et al. Field-induced polarization rotation and phase transitions in 0.70Pb (Mg1/3Nb2/3)O3− 0.30PbTiO3 piezoceramics observed by in situ high-energy x-ray scattering. Phys. Rev. B 97, 214102 (2018).
Senousy, M. S., Rajapakse, R., Mumford, D. & Gadala, M. S. Self-heat generation in piezoelectric stack actuators used in fuel injectors. Smart Mater. Struct. 18, 045008 (2009).
Lee, G. et al. Less than mm2 macrosymmetry in polarized (011)-oriented relaxor-PbTiO3 single crystals reflected in the face shear properties. Appl. Phys. Lett. 120, 042903 (2022).
Lee, G., Kim, H., Lee, S., Lee, H. & Jo, W. De-poling mechanism of alternating-current-poled Pb (Mg1/3Nb2/3)O3-PbTiO3 single crystals measured using in-situ thermally stimulated de-poling current. J. Sens. Technol. 29, 59–62 (2020).
Zhang, S. et al. Recent developments on high Curie temperature PIN–PMN–PT ferroelectric crystals. J. Cryst. Growth 318, 846–850 (2011).
Wang, Y. et al. Improved thermal stability of [001]c poled 0.24Pb(In1/2Nb1/2)O3–0.47Pb (Mg1/3Nb2/3)O3–0.29PbTiO3 single crystal with manganese doping. J. Alloy. Compd. 601, 154–157 (2014).
Zhou, Y. et al. Domain switching and polarization fatigue in rhombohedral PIN‐PMN‐PT and Mn‐doped PIN‐PMN‐PT single crystals. J. Am. Ceram. Soc. 102, 6668–6679 (2019).
Liu, X. et al. Different domain switching kinetics in tetragonal PMN-PT single crystal studied by in situ observation and current analysis. J. Eur. Ceram. 40, 2922–2928 (2020).
Chaipanich, A. & Jaitanong, N. Effect of poling time on piezoelectric properties of 0-3 PZT-portland cement composites. Ferroelectr. Lett. Sect. 35, 73–78 (2008).
Li, Q. et al. Poling engineering of (K, Na) NbO 3-based lead-free piezoceramics with orthorhombic–tetragonal coexisting phases. J. Mater. Chem. C. 5, 549–556 (2017).
Tagantsev, A. K., Stolichnov, I., Setter, N., Cross, J. S. & Tsukada, M. Non-kolmogorov-avrami switching kinetics in ferroelectric thin films. Phys. Rev. B 66, 214109 (2002).
Sun, Y., Karaki, T. & Yamashita, Y. Recent progress on AC poling of relaxor-PbTiO3 ferroelectric single crystals: a review. JPN. J. Appl. Phys. 61, SB0802 (2022).
Kim, H. et al. A review on alternating current poling for perovskite relaxor-PbTiO3 single crystals. IEEE Trans. Ultrason. Ferroelectr. Freq. Control 69, 3037–3047 (2022).
Chang, W. et al. Dielectric and piezoelectric properties of 0.7Pb(Mg1/3Nb2/3) O3−0.3PbTiO3 single crystal poled using alternating current. Mater. Res. Lett. 6, 537–544 (2018).
Wan, H. et al. The overpoling effect of alternating current poling on rhombohedral Pb (Mg1/3Nb2/3) O3-PbTiO3 single crystals. Appl. Phys. Lett. 120, 192901 (2022).
Chen, L. Phase‐field method of phase transitions/domain structures in ferroelectric thin films: a review. J. Am. Ceram. Soc. 91, 1835–1844 (2008).
Wang, J., Wang, B. & Chen, L. Understanding, predicting, and designing ferroelectric domain structures and switching guided by the phase-field method. Annu. Rev. Mater. Res. 49, 127–152 (2019).
Khakpash, N., Khassaf, H., Rossetti, G. A. Jr. & Alpay, S. P. Misfit strain phase diagrams of epitaxial PMN–PT films. Appl. Phys. Lett. 106, 082905 (2015).
Qiu, C. et al. Transparent ferroelectric crystals with ultrahigh piezoelectricity. Nature 577, 350–354 (2020).
Hu, H. L. & Chen, L. Q. Three-dimensional computer simulation of ferroelectric domain. Formation. J. Am. Ceram. Soc. 81, 492–500 (1998).
Acknowledgements
This work was primarily supported by ONR under Grant # N00014-21-1-2058, and in part supported by the National Science Foundation (NSF) through Grants: DMR 2011978 and DMR-2309184. The phase-field simulations and analysis were performed under the auspices of the National Science Foundation under DMR- 2133373 (M.H.Z and L.Q.C.) and under the U.S. Department of Energy by Lawrence Livermore National Laboratory under Contract DE-AC52-07NA27344 (B.W.).
Ethics declarations
Competing interests
The authors declare no competing interests.
Peer review
Peer review information
Nature Communications thanks the anonymous reviewer(s) for their contribution to the peer review of this work. A peer review file is available.
Additional information
Publisher’s note Springer Nature remains neutral with regard to jurisdictional claims in published maps and institutional affiliations.
Supplementary information
Rights and permissions
Open Access This article is licensed under a Creative Commons Attribution-NonCommercial-NoDerivatives 4.0 International License, which permits any non-commercial use, sharing, distribution and reproduction in any medium or format, as long as you give appropriate credit to the original author(s) and the source, provide a link to the Creative Commons licence, and indicate if you modified the licensed material. You do not have permission under this licence to share adapted material derived from this article or parts of it. The images or other third party material in this article are included in the article’s Creative Commons licence, unless indicated otherwise in a credit line to the material. If material is not included in the article’s Creative Commons licence and your intended use is not permitted by statutory regulation or exceeds the permitted use, you will need to obtain permission directly from the copyright holder. To view a copy of this licence, visit http://creativecommons.org/licenses/by-nc-nd/4.0/.
About this article
Cite this article
Kim, HP., Zhang, MH., Wang, B. et al. Electrical de-poling and re-poling of relaxor-PbTiO3 piezoelectric single crystals without heat treatment. Nat Commun 15, 6420 (2024). https://doi.org/10.1038/s41467-024-50847-3
Received:
Accepted:
Published:
DOI: https://doi.org/10.1038/s41467-024-50847-3
Subjects
No comments:
Post a Comment